- 1.1 Fluid Mechanics in Chemical Engineering
- 1.2 General Concepts of a Fluid
- 1.3 Stresses, Pressure, Velocity, and the Basic Laws
- 1.4 Physical Properties-Density, Viscosity, and Surface Tension
- 1.5 Units and Systems of Units
- 1.6 Hydrostatics
- 1.7 Pressure Change Caused by Rotation
- Problems for Chapter 1
1.3 Stresses, Pressure, Velocity, and the Basic Laws
Stresses. The concept of a force should be readily apparent. In fluid mechanics, a force per unit area, called a stress, is usually found to be a more convenient and versatile quantity than the force itself. Further, when considering a specific surface, there are two types of stresses that are particularly important.
The first type of stress, shown in Fig. 1.3(a), acts perpendicularly to the surface and is therefore called a normal stress; it will be tensile or compressive, depending on whether it tends to stretch or to compress the fluid on which it acts. The normal stress equals F/A, where F is the normal force and A is the area of the surface on which it acts. The dotted outlines show the volume changes caused by deformation. In fluid mechanics, pressure is usually the most important type of compressive stress, and will shortly be discussed in more detail.
Fig. 1.3(a) Tensile and compressive normal stresses F/A, acting on a cylinder, causing elongation and shrinkage, respectively.
The second type of stress, shown in Fig. 1.3(b), acts tangentially to the surface; it is called a shear stress τ and equals F/A, where F is the tangential force and A is the area on which it acts. Shear stress is transmitted through a fluid by interaction of the molecules with one another. A knowledge of the shear stress is very important when studying the flow of viscous Newtonian fluids. For a given rate of deformation, measured by the time derivative dγ/dt of the small angle of deformation γ, the shear stress τ is directly proportional to the viscosity of the fluid (see Fig. 1.3(b)).
Fig. 1.3(b) Shear stress τ = F/A, acting on a rectangular parallelepiped, shown in cross section, causing a deformation measured by the angle γ (whose magnitude is exaggerated here).
Pressure. In virtually all hydrostatic situations—those involving fluids at rest—the fluid molecules are in a state of compression. For example, for the swimming pool whose cross section is depicted in Fig. 1.4, this compression at a typical point P is caused by the downward gravitational weight of the water above point P. The degree of compression is measured by a scalar, p—the pressure.
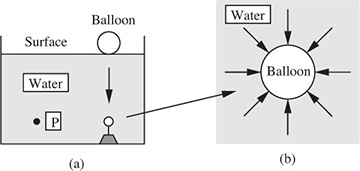
Fig. 1.4 (a) Balloon submerged in a swimming pool; (b) enlarged view of the compressed balloon, with pressure forces acting on it.
A small inflated spherical balloon pulled down from the surface and tethered at the bottom by a weight will still retain its spherical shape (apart from a small distortion at the point of the tether), but will be diminished in size, as in Fig. 1.4(a). It is apparent that there must be forces acting normally inward on the surface of the balloon and that these must essentially be uniform for the shape to remain spherical, as in Fig. 1.4(b).
Although the pressure p is a scalar, it typically appears in tandem with an area A (assumed small enough so that the pressure is uniform over it). By definition of pressure, the surface experiences a normal compressive force F = pA. Thus, pressure has units of a force per unit area—the same as a stress.
The value of the pressure at a point is independent of the orientation of any area associated with it, as can be deduced with reference to a differentially small wedge-shaped element of the fluid, shown in Fig. 1.5.
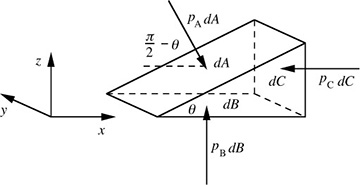
Fig. 1.5 Equilibrium of a wedge of fluid.
Due to the pressure there are three forces, pAdA, pBdB, and pCdC, that act on the three rectangular faces of areas dA, dB, and dC. Since the wedge is not moving, equate the two forces acting on it in the horizontal or x direction, noting that pAdA must be resolved through an angle (π/2 – θ) by multiplying it by cos(π/2 – θ) = sin θ:
The vertical force pBdB acting on the bottom surface is omitted from Eqn. (1.1) because it has no component in the x direction. The horizontal pressure forces acting in the y direction on the two triangular faces of the wedge are also omitted, since again these forces have no effect in the x direction. From geometrical considerations, areas dA and dC are related by:
These last two equations yield:
verifying that the pressure is independent of the orientation of the surface being considered. A force balance in the z direction leads to a similar result, pA = pB.1
For moving fluids, the normal stresses include both a pressure and extra stresses caused by the motion of the fluid, as discussed in detail in Section 5.6.
The amount by which a certain pressure exceeds that of the atmosphere is termed the gauge pressure, the reason being that many common pressure gauges are really differential instruments, reading the difference between a required pressure and that of the surrounding atmosphere. Absolute pressure equals the gauge pressure plus the atmospheric pressure.
Velocity. Many problems in fluid mechanics deal with the velocity of the fluid at a point, equal to the rate of change of the position of a fluid particle with time, thus having both a magnitude and a direction. In some situations, particularly those treated from the macroscopic viewpoint, as in Chapters 2, 3, and 4, it sometimes suffices to ignore variations of the velocity with position. In other cases—particularly those treated from the microscopic viewpoint, as in Chapter 6 and later—it is invariably essential to consider variations of velocity with position.
Velocity is not only important in its own right but leads immediately to three fluxes or flow rates. Specifically, if u denotes a uniform velocity (not varying with position):
If the fluid passes through a plane of area A normal to the direction of the velocity, as shown in Fig. 1.6, the corresponding volumetric flow rate of fluid through the plane is Q = uA.
The corresponding mass flow rate is m = ρQ = ρuA, where ρ is the (constant) fluid density. The alternative notation with an overdot,
, is also used.
When velocity is multiplied by mass, it gives momentum, a quantity of prime importance in fluid mechanics. The corresponding momentum flow rate passing through the area A is
.

Fig. 1.6 Fluid passing through an area A: (a) uniform velocity, (b) varying velocity.
If u and/or ρ should vary with position, as in Fig. 1.6(b), the corresponding expressions will be seen later to involve integrals over the area A: Q = ∫A u dA, m = ∫A ρu dA, .
Basic laws. In principle, the laws of fluid mechanics can be stated simply, and—in the absence of relativistic effects—amount to conservation of mass, energy, and momentum. When applying these laws, the procedure is first to identify a system, its boundary, and its surroundings; and second, to identify how the system interacts with its surroundings. Refer to Fig. 1.7 and let the quantity X represent either mass, energy, or momentum. Also recognize that X may be added from the surroundings and transported into the system by an amount Xin across the boundary, and may likewise be removed or transported out of the system to the surroundings by an amount Xout.
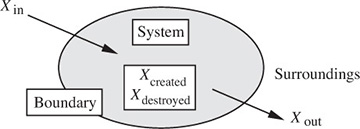
Fig. 1.7 A system and transports to and from it.
The general conservation law gives the increase ΔXsystem in the X-content of the system as:
Although this basic law may appear intuitively obvious, it applies only to a very restricted selection of properties X. For example, it is not generally true if X is another extensive property such as volume, and it is quite meaningless if X is an intensive property such as pressure or temperature.
In certain cases, where Xi is the mass of a definite chemical species i, we may also have an amount of creation or destruction
due to chemical reaction, in which case the general law becomes:
The conservation law will be discussed further in Section 2.1 and is of such fundamental importance that in various guises it will find numerous applications throughout all of this text.
To solve a physical problem, the following information concerning the fluid is also usually needed:
The physical properties of the fluid involved, as discussed in Section 1.4.
For situations involving fluid flow, a constitutive equation for the fluid, which relates the various stresses to the flow pattern.